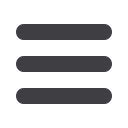
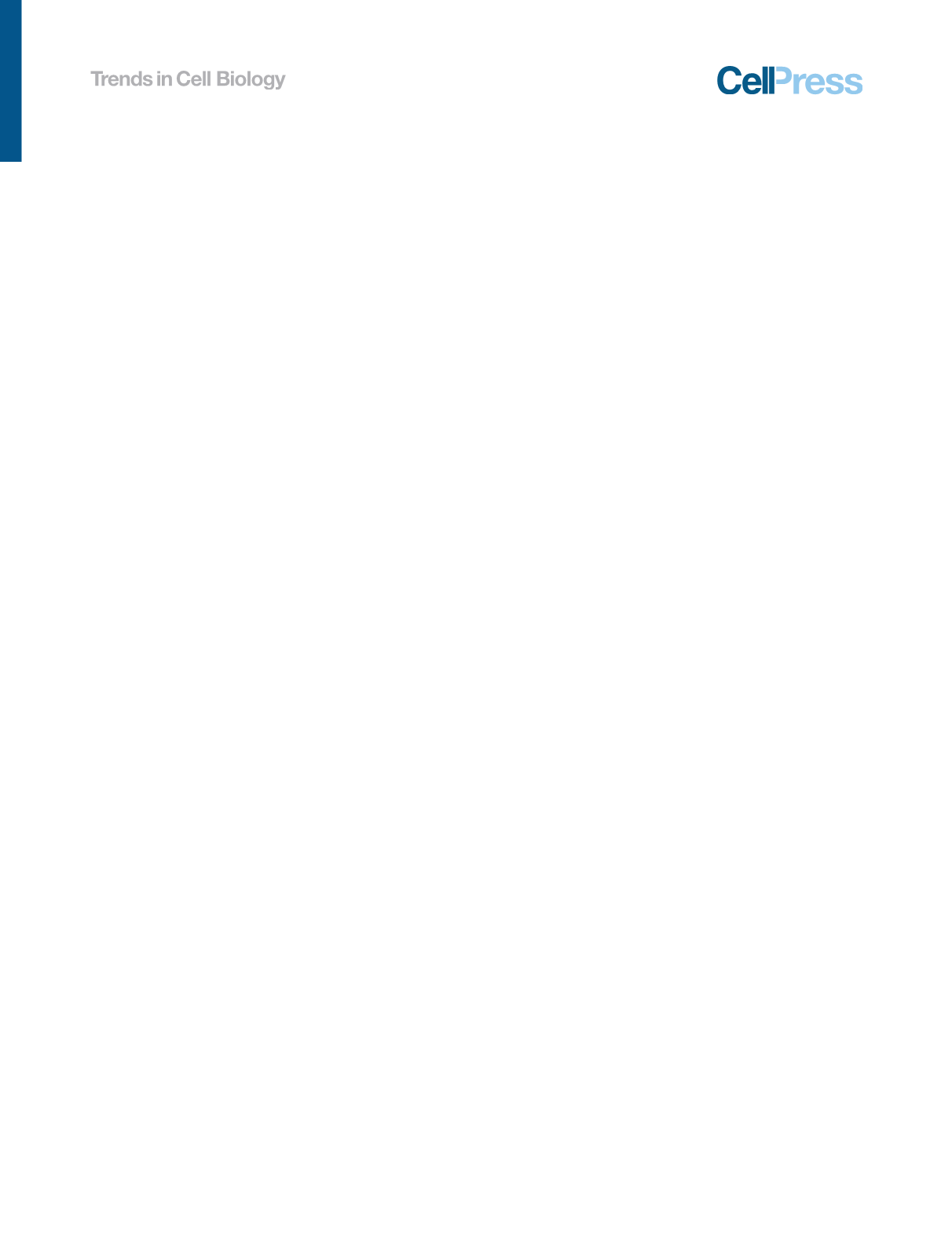
[35,36], which is indicative of a mechanism for targeting and modulating RNAs in cells. The
recent discovery of the protein Cpf1 from the
Prevotella
and
Francisella
-1 type V CRISPR
showed that Cpf1 uses a short crRNA without a tracrRNA for RNA-guided DNA cleavage
[37
–
40]. Both biochemical and cell culture work showed that Cpf1-mediated genome target-
ing is effective and speci
fi
c, comparable with the
S. pyogenes
Cas9. The type VI-A CRISPR
effector C2c2 from the bacterium
Leptotrichia shahii
is a RNA-guided RNase that can be
programmed to knock down speci
fi
c mRNAs in bacteria [41]. These results broaden our
understanding of the diversity of natural CRISPR
[5_TD$DIFF]
/Cas systems, which also provide a func-
tionally diverse set of tools.
Other enzymatic domains can also be harnessed for genome editing. For example, instead of
using the endonuclease activity of Cas9, a mutation in one nuclease domain of Cas9 can create
a nickase Cas9 (nCas9) that can cleave one strand of DNA [42]. With a pair of sgRNAs, the
speci
fi
city of genome editing could be enhanced by using a pair of nCas9s that target each
strand of DNA at adjacent sites. Furthermore, recent work demonstrated that a Cas9-fused
cytidine deaminase enzyme allowed for direct conversion of a C to T (or G to A) substitution [43].
In this work, fusing the nuclease-deactivated dCas9 or the nCas9 with a cytidine deaminase
domain corrected point mutations relevant to human disease without DSBs; therefore, avoiding
NHEJ-mediated indel formation.
Applications of CRISPR/Cas9 for Cell Biological Studies
The CRISPR/Cas9 technology has accelerated the discovery and mechanistic interrogation of
the genome and organelles in diverse types of cell and organism. Some examples of utilizing
CRISPR/Cas9 for studying cellular organelles are summarized in Table 1 and Figure 3. Beyond
using CRISPR/Cas9 as a gene-editing tool, we describe the development of CRISPR/Cas9 as a
versatile toolkit for transcriptional control and epigenetic regulation, and highlight its utilities for
large-scale genetic screens, generation of animal models, genomic imaging, and lineage tracing
(Figure 2).
Transcriptional Regulation of the Genome with CRISPR/dCas9
The nuclease-dead dCas9 has provided a broad platform for programming diverse types of
transcriptional or epigenetic manipulation of the genome, without altering the genome
sequence. In brief, dCas9 was created by introducing point mutations into the HNH and RuvC
domains to eliminate endonuclease activity [44]. This repurposed protein became a RNA-guided
DNA-binding protein. In bacteria, the dCas9 protein was suf
fi
cient to induce strong sequence-
speci
fi
c gene repression, simply by sterically hindering the transcriptional activity of RNA
polymerase [44,45]. In eukaryotic cells, fusing dCas9 to transcriptional effector proteins allowed
for more ef
fi
cient RNA-guided transcriptional modulation for both gene interference (CRISPRi)
and activation (CRISPRa) [12,46
–
48].
By fusing dCas9 to transcriptional repressors, such as the Kruppel-associated box (KRAB)
domain, CRISPRi can ef
fi
ciently repress coding and noncoding genes, such as miRNAs and
large intergenic noncoding RNAs (lincRNAs) in mammalian cells [46,47,49,50]. Compared
with complete loss-of-function using Cas9, CRISPRi can use different sgRNAs that bind to
different genomic loci for tunable and titratable gene repression [47]. While complete
knockout is useful for studying gene function in many cases, tunable repression of a gene
to different levels offers advantages when knocking out a gene leads to lethality of cells or an
organism [45].
Earlier work using dCas9 fused to a peptide containing multiple VP16 domains (VP64 or VP128)
could only activate endogenous genes mildly [46,51,52]; therefore, several strategies have been
developed to improve CRISPRa ef
fi
ciency. These include recruiting multiple copies of the VP64
878
Trends in Cell Biology, November 2016, Vol. 26, No. 11