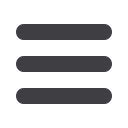
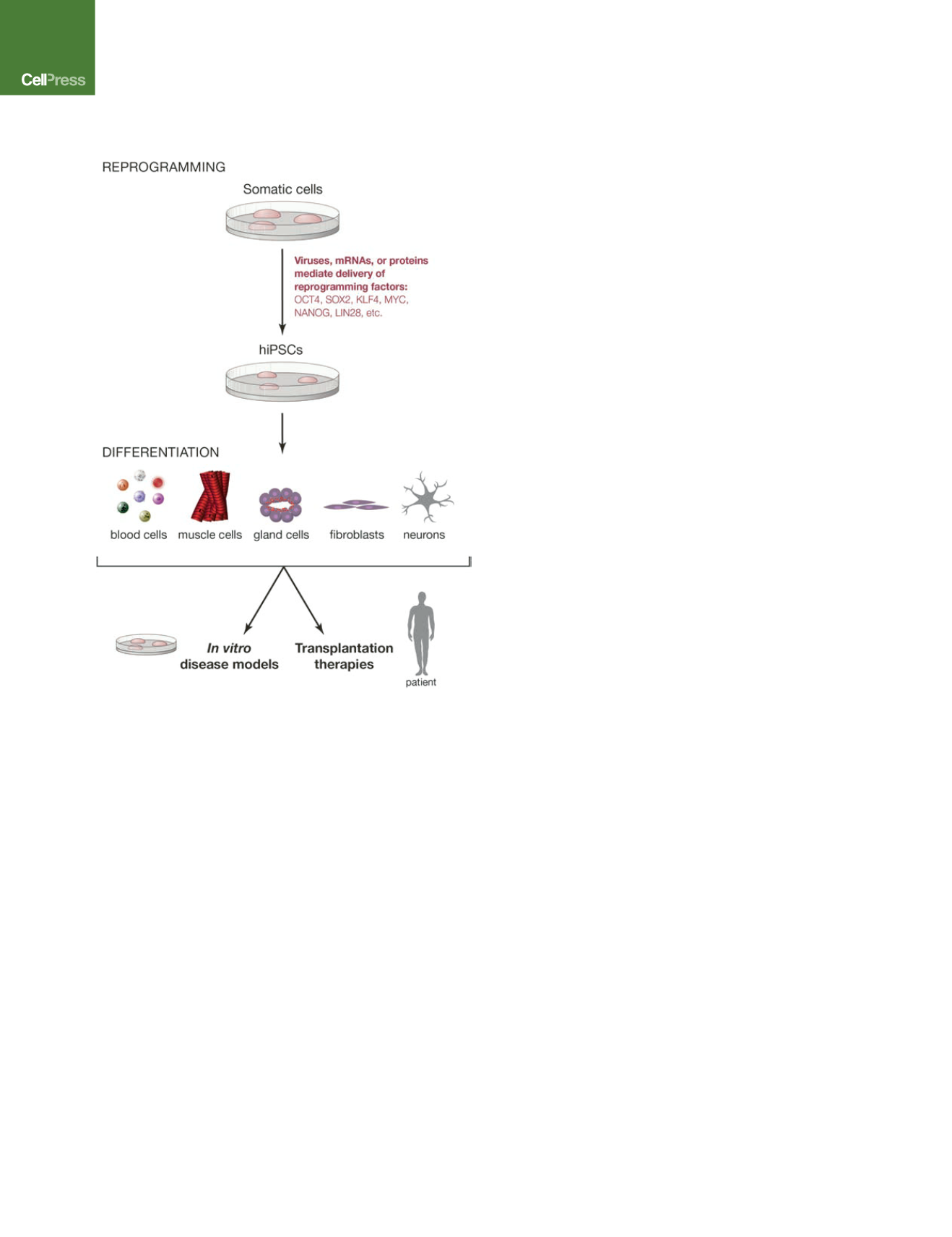
that is captured in vitro from the outgrowth of the inner cell mass,
where hPSCs cultured under standard conditions represent
a later epiblast-like pluripotent state (Brons et al., 2007; Tesar
et al., 2007; Theunissen et al., 2014) (reviewed in Nichols and
Smith, 2009).
Proof-of-concept experiments with cells differentiated from
hESCs suggested that PSCs could be a source for cell replace-
ment transplantation therapies and could provide a model
system to understand early human development and cellular dif-
ferentiation. However, ethical concerns, limited access to em-
bryos, and the possibility of immune rejection were roadblocks
that impeded the promise of hESCs.
In 2006 the ‘‘Yamanaka experiments’’ made the ethical debate
about PSC research largely obsolete, as they established a
robust method to derive human pluripotent cells without the
use of human embryos. Furthermore iPSC technology promised
to solve complications that were anticipated from immune rejec-
tions of heterologous hESC-derived tissues, as it would allow the
generation of patient-specific autologous pluripotent cells and
derived tissue. The race to perform the key functional follow-
up experiments began immediately. For the mouse system it
was essential to establish that iPSCs could pass the most strin-
gent test for pluripotency: germline transmission (Maherali et al.,
2007; Okita et al., 2007; Wernig et al., 2007) and tetraploid
complementation (Kang et al., 2009; Zhao et al., 2009).
For the human system the initial question was whether the
same set of factors capable of reprogramming mouse cells
would also work for human cells (Takahashi et al., 2007). Yama-
naka and Takahashi quickly demonstrated that their factors also
worked in human cells (Takahashi et al., 2007). However, addi-
tional experiments over that last 10 years in mouse and human
cells also revealed that other sets of transcription factor combi-
nations can be equally potent in reprogramming cells to a plurip-
otent state, providing valuable insights into the transcriptional
pluripotency networks and how cells establish pluripotency
(Buganim et al., 2012; Apostolou and Hochedlinger, 2013; Park
et al., 2008; Takahashi and Yamanaka, 2015; Yu et al., 2007).
For the anticipated clinical application of iPSCs, it was impor-
tant to demonstrate that reprogramming could be achieved
without stably integrating the KSOM factors into the genome of
the somatic cell. Such factor-free iPSCs were generated by inde-
pendent methods such as the excision of reprogramming factors
using the Cre/LoxP (Soldner et al., 2009) or the piggyBac system
(Kaji et al., 2009; Woltjen et al., 2009) by avoiding integration of
the reprogramming factors altogether by using non-integrating
viruses (Fusaki et al., 2009), episomal vectors (Yu et al., 2009),
or direct transfection of the reprogramming factors as either
mRNA (Warren et al., 2010) or protein (Kim et al., 2009). Initially,
human cell reprogramming was quite inefficient compared to
mouse cells, and thus several technical improvements were
made to optimize hiPSC reprogramming protocols, culture con-
ditions, and iPSC characterization procedures to test for the
pluripotency of newly isolated iPSCs. Eventually, these optimiza-
tions made iPSC technology increasingly more accessible to
laboratories without previous stem cell experience and are
now so streamlined that iPSC derivation, maintenance, and dif-
ferentiation are widely used research tools in all aspects of
biomedical research. In addition, efficient and robust reprogram-
ming techniques provided insight into the mechanistic steps of
reprogramming and the order of events involved in reverting
the epigenome from a differentiated to a pluripotent state.
A detailed understanding of the forces at work is necessary to
answer the key questions of whether the reprogramming of hu-
man cells results in a cell state that is equivalent to hESCs or
whether iPSCs retain to some extent an epigenetic memory
(Kim et al., 2010; Polo et al., 2010). For example, do iPSCs
derived from liver cells retain some characteristics of liver cells
and do they preferentially differentiate into liver tissue relative
to other cell types? Tetraploid complementation and germline
transmission experiments gave the clear answers that mouse
iPSCs were fully reprogrammed to pluripotency. However these
tests are not available for hiPSCs. Moreover, it is not clear to
what extent the mouse iPSC’s epigenome is reset during the re-
programming process and how much of the resetting occurs
in vivo or when the cells pass through the germline. It is not sur-
prising that early cellular stages of the reprogramming process
will show epigenetic differences, yet all these differences even-
tually will converge on the same pluripotent cell state as ESCs.
Thus, it is interesting to further examine the level and func-
tional relevance of epigenetic memory; yet it seems that such
Figure 1. Overview of the iPSC Technology
Patient cells can be reprogrammed into iPSCs using optimized reprogram-
ming protocols that involve small molecules, microRNAs, and combinations of
reprogramming factors. iPSCs can be differentiated into somatic cells that
could be used either in transplantation therapies or alternatively to model
human diseases.
574
Cell Stem Cell
18
, May 5, 2016
Cell Stem Cell
Review