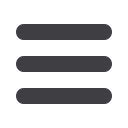
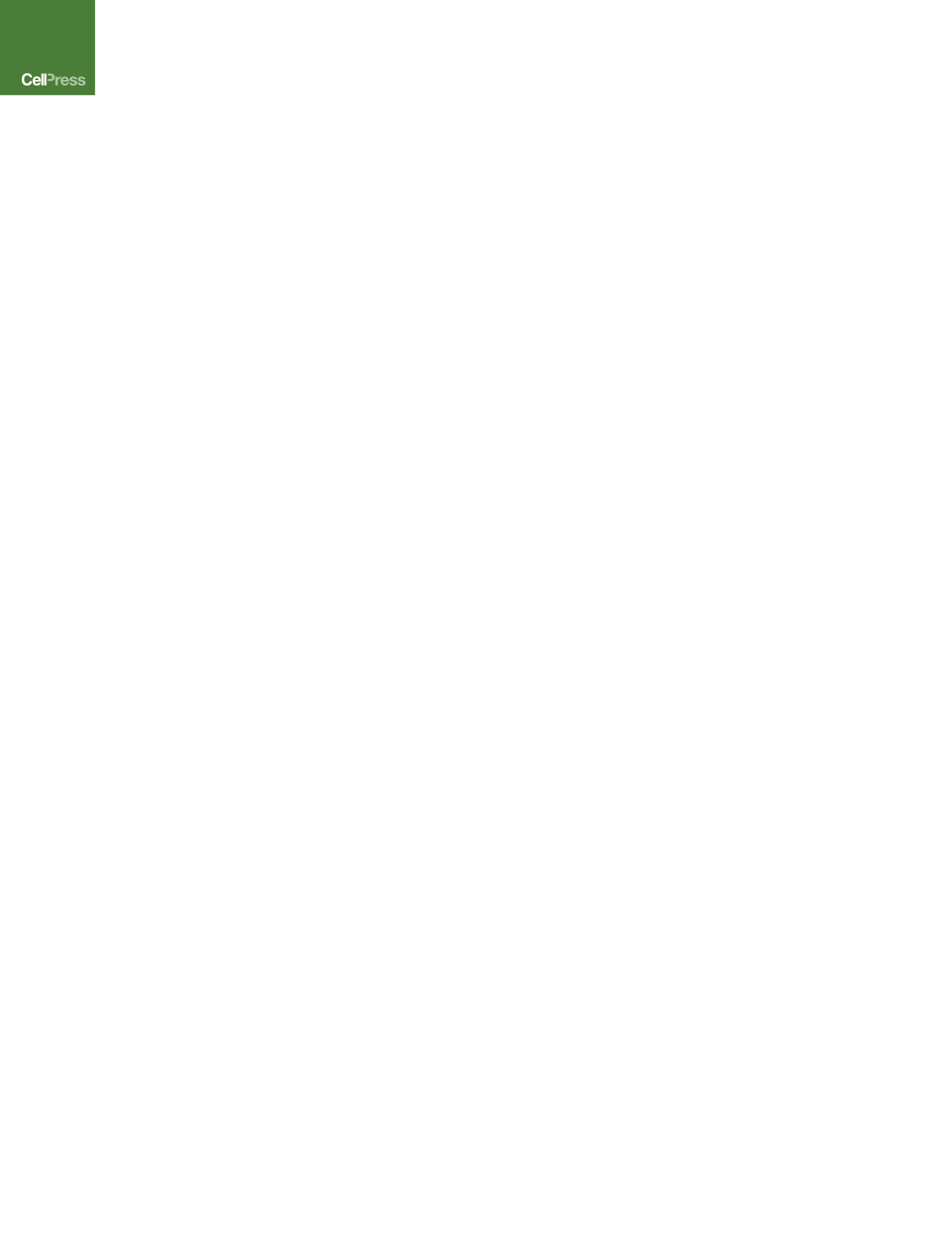
Most experiments that have detected significant off-targets
have been performed in cancer cells, which may have altered
repair pathways that could affect recombination (Fu et al.,
2013; Hsu et al., 2013). In contrast, experiments in whole organ-
isms such as mice (Wang et al., 2013), primates (Niu et al., 2014),
Zebrafish (Auer et al., 2014), or
C. elegans
(Dickinson et al., 2013)
reported off-target frequencies that were low or not detectable,
consistent with high specificity of the CRISPR/Cas9-mediated
gene targeting. It is also possible that in non-transformed cells
off-target cleavages are efficiently counter-selected by the
endogenous DNA-damage response. As hPSCs are primary
cells with genetically intact check-points it seems possible that
off-targets will accumulate less frequently in hPSCs than has
been observed in cancer cells. To address this it will be important
to determine to what extent off-targets are the result of impaired
checkpoint control of cancer cells and whether there are specific
cell types and conditions that are predisposed for the accumu-
lation of off-targets. Data from conventional whole-genome
sequencing of hPSCs exposed to Cas9 have so far been limited
and do not yet fully address the issues due to small sample sizes
(Park et al., 2015; Smith et al., 2014).
Understanding how to avoid off-target SSN modification is of
particular concern for the eventual clinical application of edited
cells. For basic research, however, it seems that the necessary
experiments are readily available to control for the effects of
eventual off-target action of SSNs. Experiments to adequately
address off-target concerns include: (1) the use of several inde-
pendent guide RNAs to generate a mutant cell line, (2) comple-
mentation of loss-of-function phenotypes, and (3) secondary
editing of the mutant cell line to revert the mutation to a WT allele
followed by confirmation of phenotypic rescue.
Large-Scale Screens, Epigenetic Editing, and Other
Applications for Cas9 in iPSCs
In addition to allowing the easy, fast, and inexpensive editing of
hPSCs, the advent of Cas9 as a programmable DNA-binding
protein allowed the development of forward genetics methodol-
ogies that were previously not readily available in hESCs. It is
trivial to multiplex guide RNA synthesis, which allows the gener-
ation of large barcoded libraries of sgRNAs with several-fold
coverage of every gene in the human genome. These libraries
can be employed in loss-of-function screens, for example, to
identify gene products that are required for drug resistance or
the mediation of viral cell death (Gilbert et al., 2014; Hart et al.,
2015; Parnas et al., 2015; Shalem et al., 2014; Shi et al., 2015;
Wang et al., 2015a; Wang et al., 2014a; Zhou et al., 2014)
(Figure 2). Most of the experiments that employ genome-wide
screens have been done in cancer cells that can be expanded
to accommodate the large numbers of cells that are required
to perform these types of genome-wide screens. For the general
implementation of these screening approaches in hPSCs or cells
differentiated from hPSCs, it will be important to develop
protocols that allow the expansion of these cells into large
homogenous populations that in turn allow robust selection or
enrichment for cellular phenotypes.
It is worth mentioning that the combination of iPSCs and
genome editing has not only become a game changer for our ap-
proaches to human disease modeling, but it also provides an
unprecedented opportunity to study the fundamental principles
of cell biology. Previously, cell biologists mostly used aberrant
cancer cell lines with often unstable and poorly defined genomes
to describe human cellular behavior. This is mainly because hu-
man cancer cells presented the only reliable source of human
immortal cells that could be expanded sufficiently to facilitate
biochemical and genetic experimentation and could be indefi-
nitely propagated, frozen, shipped, and shared between labs.
This monopoly of cancer cells as a model system was broken
with the advent of hiPSCs and the general availability of hPSCs.
Like cancer cells, hPSCs are immortal, but they do not suffer
from the disadvantages of the pathologically altered genomes
of cancer cells and yet they still retain the capacity to differentiate
into any cell type of interest. The combination of hPSCs with the
power of genome editing can now be used to study specific
aspects of human cell biology. Exploiting this potential will
be particularly important in areas of research where funda-
mental biological processes, such as tumor suppression, cellular
immortality, or neuronal biology, diverge between human and
other species. Efforts, such as the one launched by the Allen
Institute for Cell Science to generate an industrial-scale library
of characterized iPSCs that will be used to create a visual,
animated model of the cell, suggest that iPSCs will soon replace
cancer cells as a model system for basic cell biology (Callaway,
2014).
In the same way that iPSC technology had broad impacts far
beyond regenerative medicine and disease modeling, the impact
of the discovery of CRISPR/Cas9 on hPSCs is not only its ability
to act as an SSN. Catalytically inactive forms of Cas9 (dCas9)
have been successfully derived by fusions with functional pro-
teins that bind specific loci, or the activation or repression of
gene activity at the target site (Chen et al., 2013; Gilbert et al.,
2014; Konermann et al., 2015; Mandegar et al., 2016; Tanen-
baum et al., 2014) (CRISPRa and CRISPRi, Figure 2). Some
of these platforms have been successfully implemented for
genome-wide screens and the manipulation of hPSCs. As
demonstrated for TALE proteins and zinc finger DNA binding do-
mains, the range of Cas9 could be extended in the future to also
methylate or demethylate DNA or histones/chromatin at precise
locations in the genome (Maeder et al., 2013; Meister et al.,
2010). Moreover, dCas9 fused to fluorescent reporters has
been developed to indicate nuclear organization by visualizing
individual genomic loci (Chen et al., 2013; Gilbert et al., 2014; Ta-
nenbaum et al., 2014). It is exciting that more applications are
being developed; recently, Cas9 has been programmed to target
RNA in vitro and in vivo (O’Connell et al., 2014; Nelles et al.,
2016), raising the possibly that it could be used to better under-
stand the transcriptome in addition to the genome.
Arguably the most far-reaching consequence of CRISPR/Cas9
gene targeting is the potential to edit the germline. Because gene
editing by homologous recombination is inefficient, cells carrying
the desired targeting event need to be selected in culture. Thus,
germline modification in the past was restricted to mice as
chimera-competent ESCs are not available in other species.
Because CRISPR/Cas9 gene editing is so efficient, it requires
no selection for the desired targeting events, rendering ESCs su-
perfluous for the generation of mutant animals. CRISPR/Cas9
enabled gene editing in the zygote and was used to efficiently
generate animals carrying defined mutations in multiple species
including fish,
Drosophila
, mice, and primates (Bassett et al.,
2013; Chang et al., 2013; Gratz et al., 2013; Hwang et al.,
580
Cell Stem Cell
18
, May 5, 2016
Cell Stem Cell
Review