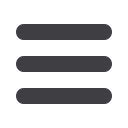
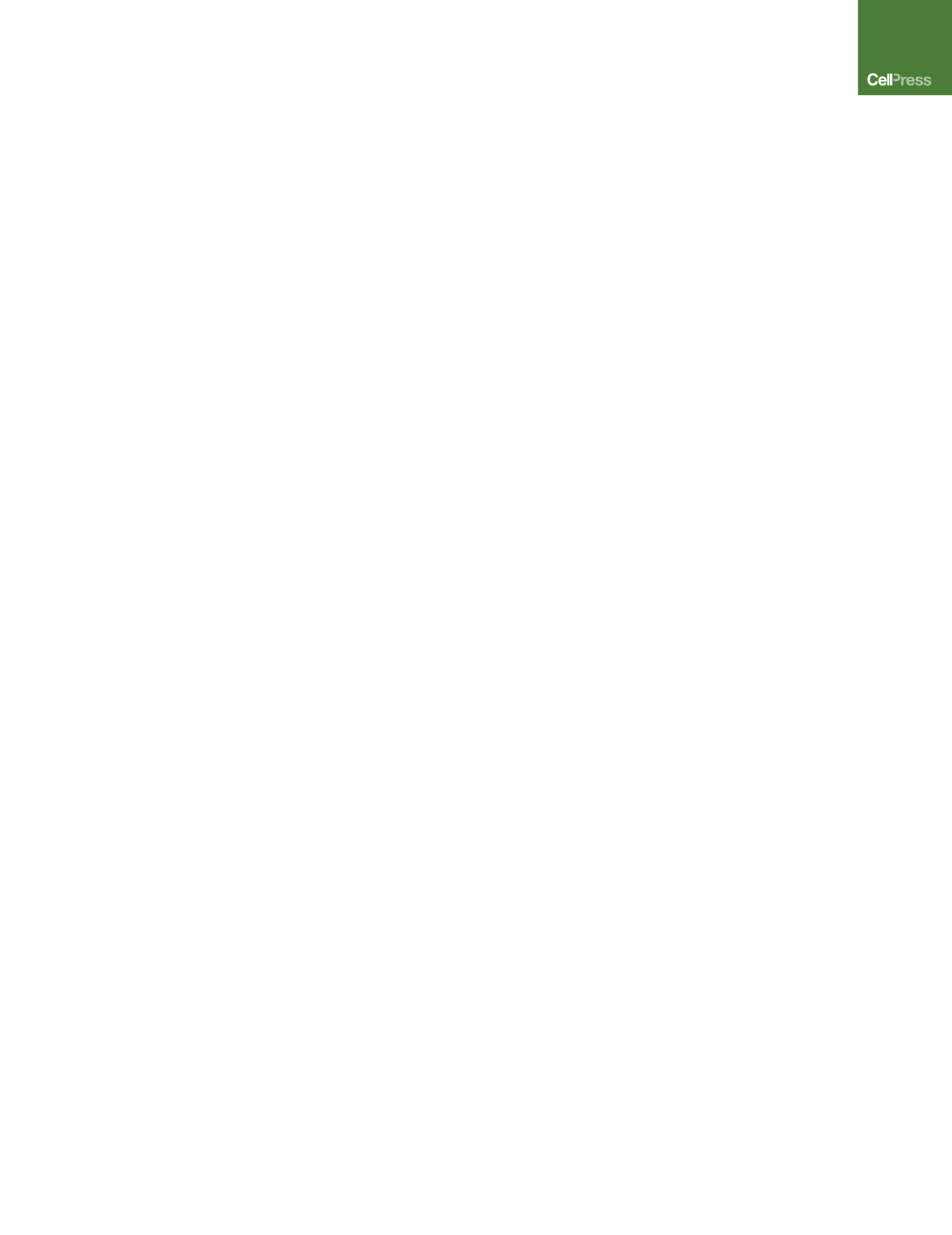
epigenetic differences in cellular state are at best small and over-
shadowed by differences caused by the reprograming method of
choice, cell selection during propagation, culture conditions, and
more importantly genetic background of the parental somatic
cell (Guenther et al., 2010; Kytta¨ la¨ et al., 2016; Rouhani et al.,
2014). For example, it has been demonstrated that the epige-
netic memory, i.e. epigenetic characteristics reflecting the state
of the donor cells seen initially in the iPSCs, is lost upon pro-
longed cell passages, suggesting that this donor-cell-specific
memory may be of little functional relevance (Polo et al., 2010).
The most attractive application of the iPSC technology is that it
allows the isolation of patient-derived cells that carry all genetic
alterations that cause the particular disease. Thus, these cells
provide an experimental system to study pathogenesis of the
disease in an in vitro system and to possibly devise therapeutic
strategies (Robinton and Daley, 2012). Importantly, the iPSC
technology allows comparison of the neuroanatomical features
and physiology of the iPSCs to the clinical features of the donor
patient.
The Power and Limitations of iPSCs
In addition to the prospect of future iPSC-based cell replacement
therapies, the ability to derive iPSCs from patients’ cells had a
striking effect on human disease modeling. Some of the most
remarkable advances were made in diseases such as neurode-
generative diseases that are only partially recapitulated in animal
models. Here, iPSC technology was particularly transformative,
as it made it possible to study the effects of familial monoallelic
diseases as well as complex idiopathic diseases in the context
of patient-derived neurons and tissue, systems that were previ-
ously not readily available for experimental investigation. For
example, studying dopaminergic neurons differentiated from
Parkinson patient-derived iPSCs yielded insights into the
molecular causes of the disease and the identification of cellular
stressors that might exacerbate the phenotype (Soldner et al.,
2011; Soldner and Jaenisch, 2012);. As a result of such advances,
iPSC-based and primary tissue culture systems have largely
replaced previous experimental systems that studied human ge-
netic diseases using overexpression studies in cancer cell lines.
Indeed, the number of human diseases modeled in culture using
patient-derived iPSCs (‘‘disease in the dish’’) is growing rapidly
(summarized in Avior et al., 2016; Sterneckert et al., 2014).
While the approach of studying human disease in the disease-
relevant cell type resulted in many success stories and insights,
several challenges of iPSC disease modeling quickly became
apparent. For one, it became evident that many protocols that
were developed for the differentiation of hPSCs into functional
tissue resulted in embryonic rather than adult human cell types
(Bedada et al., 2015; Forster et al., 2014; Hrvatin et al., 2014;
Spence et al., 2011; Takebe et al., 2013). This observation might
not pose a problem for studies that aim to recapitulate cell-
autonomous defects of developmental diseases that likely will
become apparent after a few weeks of in vitro differentiation.
However, iPSC differentiation experiments that aim to under-
stand human disease and pathologies within the context of the
adult or as a function of human aging suffer from a lack of cellular
maturity as well as a relatively short time span limited by culture
conditions. One approach to increase the maturity of in vitro cell
systems and to mimic cellular aging is to expose these cells to
stressors that are associated with aging (Miller et al., 2013;
Studer et al., 2015). Significant progress has also been made
to current strategies of investigating cell non-autonomous bio-
logical problems, including the development of co-culture exper-
iments and protocols to differentiate hPSCs into tissue stem
cells and organoid cultures. Organoid cultures are small func-
tional tissue units composed of several distinct cell types that
can be maintained and used to recapitulate features of tissues
rather than that of individual cell types in vitro (Lancaster et al.,
2013; Sato et al., 2011; Sato et al., 2009) (reviewed in Lancaster
and Knoblich, 2014; Sato and Clevers, 2013).
An important and often ignored challenge of iPSC technology
is the variability between individual iPSC lines in their potential to
differentiate into functional cells of a given lineage. This variation
between cell lines is unpredictable and mostly caused by genetic
background differences as well as the reprogramming history of
a given cell line. Thus, in efforts to model a disease, detection of
small phenotypic differences between cells differentiated from a
patient or control iPSCs may not reveal a disease-relevant
phenotypic difference but rather reflect the system’s immanent
variation between individual iPSC lines (Soldner and Jaenisch,
2012). The generation of isogenic pairs of disease-specific and
control iPSCs that differ exclusively at the disease-causing
mutation has been used to control for the variation and has led
to defining subtle disease-relevant differences in monogenic
diseases (Soldner et al., 2011). The problem is, however, exacer-
bated when studying more clinically important sporadic or poly-
genic diseases where low-effect-size, disease-causing loci are
defined by genome-wide association studies (GWASs). Since
phenotypic differences would be expected to be small, the use
of isogenic pairs of disease-specific and control cells would be
even more important. Finally, ongoing efforts to learn about hu-
man genetic variation by studying dozens or even hundreds of
iPSC lines derived from healthy donors may give little interpret-
able information because of the unpredictable system-inherent
phenotypic variability between individual iPSC lines (differing in
millions of SNPs within each genome) and experimental varia-
tions in their differentiation. Making isogenic iPSC controls by
genome editing that differ only in a single or a few SNPs could
reduce variations due to genomic variability.
The challenge associated with the genetic variability of hPSCs
is compounded by another remarkable difference between
mouse and human PSCs: the striking resilience of hPSCs to con-
ventional gene targeting approaches. This dearth of genetic con-
trol in hPSCs prevented genetic experiments that were consid-
ered standard in mESCs. Nevertheless conventional gene
targeting has been accomplished in hPSCs (Zwaka and Thom-
son, 2003). Protocols for conventional gene targeting have
been optimized to modify hPSCs (Costa et al., 2007; Davis
et al., 2008a; Irion et al., 2007; Ruby and Zheng, 2009) and
have been successfully used to establish hPSC models for hu-
man disease such as Lesch-Nyhan syndrome (Urbach et al.,
2004). Moreover, this approach has been used to correct the dis-
ease-causing mutation with ornithine-d-aminotranferase that is
mutated in patients with gyrate atrophy (Howden et al., 2011)
or to alter the amount of disease-causing CAG repeat expan-
sions in the huntingtin gene of patient-specific iPSCs (An et al.,
2012). Furthermore, these protocols have been used to generate
linage reporters for genes such as MIXL and Olig2 to study cell
Cell Stem Cell
18
, May 5, 2016
575
Cell Stem Cell
Review