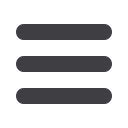
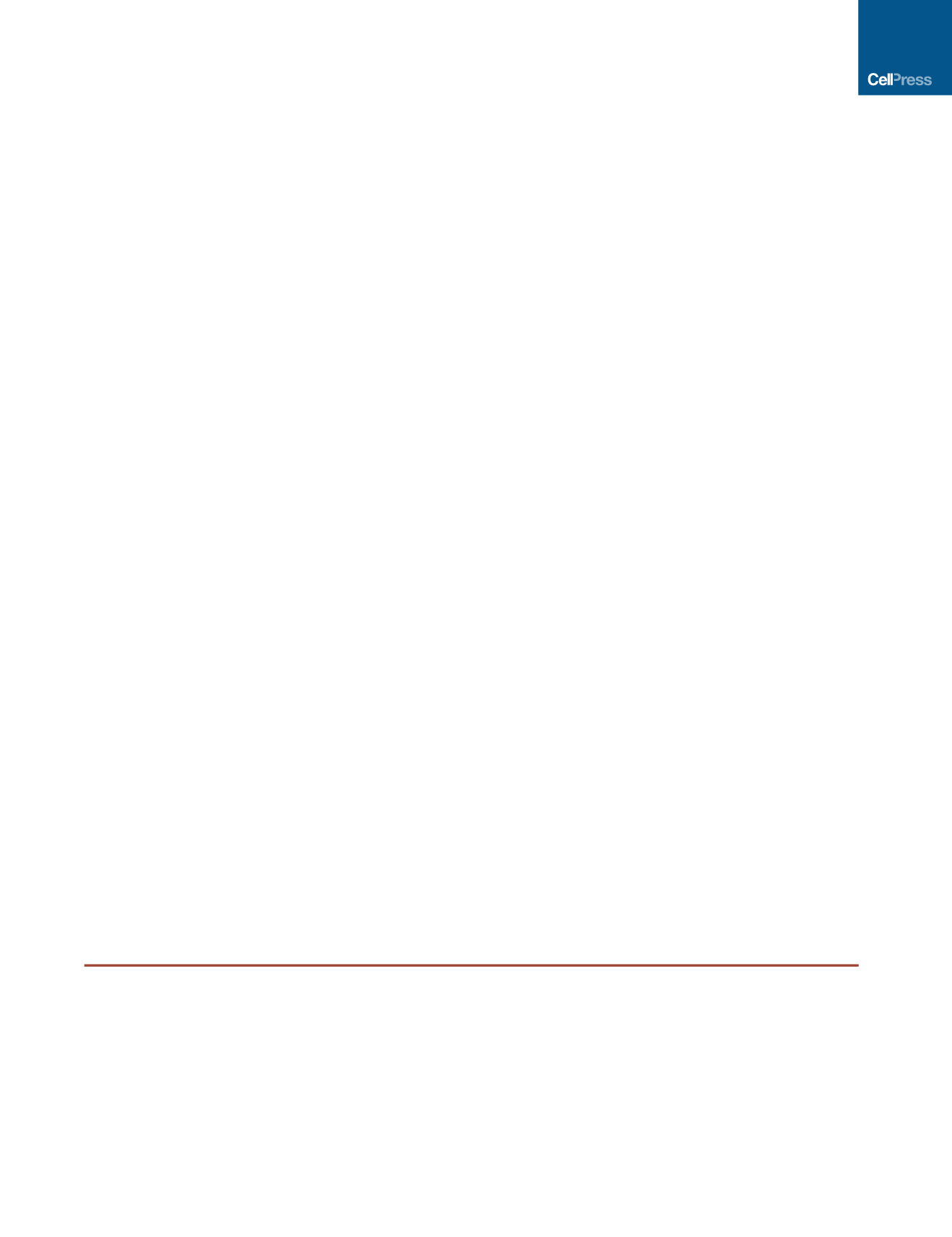
deletion that encompasses the majority of DMD mutations,
this approach is optimized for future clinical studies. It would
be unreasonable to design, validate, and evaluate off targets
for every new CRISPR pair tailored for each individual patient.
Additionally, CRISPR/Cas9 is advantageous over exon skip-
ping, as it results in permanent restoration of the reading
frame as opposed to transient effects on RNA splicing. Previ-
ously, Li et al. (2015) used CRISPR/Cas9 to induce exon skip-
ping, frameshifting, or exon knockin to restore dystrophin in a
DMD hiPSC line with an exon 44 deletion; however, their plat-
form is only applicable to 3%–9% of DMD patients (Bladen
et al., 2015), and two of their strategies relied on the creation
of indels, which would be difficult to apply consistently to
each patient. While Ousterout et al. deleted exons 45–55,
they removed significantly less of the intervening region
(336 kb) and thus their approach would cover fewer patient
mutations within the hotspot region. This is because many
mutations extend into the intronic region; thus, by designing
gRNAs that encompass more of the intron, our platform is
applicable to more patients.
Another benefit of using this platform to delete a large portion
of
DMD
, as opposed to single exons, is the known correlation of
DYS
D
45–55
with a mild BMD phenotype. Large deletions in the rod
domain of dystrophin often produce a more functional (more like
wild-type) protein, than even very small deletions (Harper et al.,
2002). Larger deletions, which remove hinge III (exons 50–51),
are believed to lead to a milder BMD phenotype than smaller de-
letions, or those that retain hinge III (Carsana et al., 2005). Thus,
in many cases larger deletions are more therapeutically benefi-
cial than smaller ones, due to the way they affect the secondary
structure of the protein.
In summary, we have developed a potentially therapeutic
gene editing platform for DMD to permanently restore the dys-
trophin reading frame in multiple patient-derived hiPSCs. Our
approach using CRISPR/Cas9 and NHEJ deletes up to 725
kb of
DMD
encompassing exons 45–55 and restores dystro-
phin protein function in both cardiomyocytes and skeletal mus-
cle cells derived from reframed hiPSCs. A current limitation of
this platform is that clinical protocols still need to be developed
that allow rapid clonal line derivation and the utilization of
hiPSC-derived cardiac and skeletal muscle progenitors com-
bined with gene correction. Alternatively, CRISPR/Cas9 to
restore the reading frame in DMD mouse models has been
delivered directly in vivo (Long et al., 2016; Nelson et al.,
2016; Tabebordbar et al., 2016). Thus, applications of this plat-
form in the future will allow for the development of an in situ
gene strategy or ex vivo gene correction followed by autolo-
gous cell transplantation, either of which offers tremendous po-
tential for DMD.
EXPERIMENTAL PROCEDURES
Differentiation of hiPSCs to Skeletal Muscle Cells and
Cardiomyocytes
Skeletal muscle differentiation from hiPSCs was induced using OE of a tamox-
ifen inducible MyoD-ERT lentivirus or an adapted 50 day directed differentia-
tion protocol where NCAM
+
HNK1 cells underwent fluorescence-activated
cell sorting at day 50. Cardiomyocytes were derived through aggregates
over 30 days. See Supplemental Experimental Procedures.
Engraftment into Immunodeficient Mice
NSG immunodeficient mice (Jackson Laboratory) were crossed to mdx
scid
mice (Jackson Laboratory) to generate NSG-mdx mice (see Supplemental
Experimental Procedures). Five- to seven-week-old NSG-mdx mice were
pretreated with 50
m
l of 10
m
M cardiotoxin (Sigma-Aldrich) injected into the
right TA 24 hr prior to engraftment. For MyoD OE cells, 100
m
l of 5 mg/ml
tamoxifen (Sigma-Aldrich) was i.p. injected for 5 days beginning on the day
prior to engraftment. 1
3
10
6
cells in HBSS were injected intramuscularly
and the TA was harvested after 30 days. See Supplemental Experimental
Procedures.
Hypo-osmotic Stress CK Release Assay
Terminally differentiated skeletal muscle cells and cardiomyocytes plated in
duplicate were stressed by incubation in hypo-osomolar solutions ranging
from 66 to 240 mosmol (see Supplemental Experimental Procedures) for
20 min at 37 C. CK was measured in triplicate from the supernatant and cell
lysate with the Creatine Kinase-SL kit (Sekisui Diagnostics) according to the
manufacturer’s instructions.
SUPPLEMENTAL INFORMATION
Supplemental Information for this article includes four figures, two tables and
Supplemental Experimental Procedures and can be found with this article on-
line at
http://dx.doi.org/10.1016/j.stem.2016.01.021.AUTHOR CONTRIBUTIONS
Conceptualization, Methodology, Writing–Original Draft, Visualization, Proj-
ect Administration, C.S.Y., M.J.S., and A.D.P.; Validation, C.S.Y., M.R.H.,
and N.V.E.; Formal Analysis, C.S.Y.; Investigation, C.S.Y., M.R.H., N.V.E.,
H.N., M.J., S.Y., S.K., C.K.-C., and D.W.; Resources, A.N., S.F.N., M.C.M.,
M.J.S., and A.D.P.; Writing–Review and Editing, C.S.Y., M.R.H., N.E.V.,
S.K., C.K.-C., D.B.K., A.N., S.F.N., M.C.M., M.J.S., and A.D.P.; Supervision,
J.A.Z., D.B.K., A.N., M.C.M., M.J.S., and A.D.P.; Funding Acquisition, M.J.S.
and A.D.P.
(B) Fold change in expression of miR31 measured by ddPCR in myotubes derived from out-of-frame or reframed hiPSCs by MyoD OE, normalized to wild-type
(CDMD 1002). Data are presented as average ± SD.
(C) Western blots of cell extracts probed with anti-
b
-dystroglycan. Extracts were from out-of-frame and reframed skeletal muscle myotubes derived by MyoD OE.
HSMM was used as a positive control. Samples were also probed with anti-MyHC as a loading control (bottom panel).
(D) Immunocytochemical staining of MyHC (red) and
b
-dystroglycan (green), a component of the DGC, in wild-type (CDMD 1002), out-of-frame (CDMD 1006), or
reframed (CDMD 1006-1) skeletal muscle myotubes. Inset depicts zoomed-in region defined by the white box. Scale bar, 50
m
m.
(E) Assessment of human dystrophin restoration in wild-type (CDMD 1002), out-of-frame (CDMD 1003), and reframed (CDMD 1003-49) MyoD OE cells engrafted
into the TA of NSG-mdx mice. Engrafted human cells were identified by co-immunostaining for human spectrin and lamin A/C (shown in red). Positive staining for
human dystrophin is shown in green and all fibers are shown using laminin (gray). All sections were stained with DAPI (blue) to identify nuclei. Scale bar, 100
m
m.
(F) Assessment of
b
-dystroglycan restoration in human fibers from wild-type (CDMD 1002), out-of-frame (CDMD 1003), and reframed (CDMD 1003-49) MyoD OE
cells engrafted into the TA of NSG-mdx mice. Engrafted human cells were identified by co-immunostaining for human spectrin and lamin A/C (shown in red).
Positive staining for dystrophin is shown in gray and
b
-dystroglycan is shown in green. All sections were stained with DAPI (blue) to identify nuclei. Cell order is the
same as noted in (E). Scale bar, 20
m
m.
See also Figures S4E and S4F.
Cell Stem Cell
18
, 533–540, April 7, 2016
ª
2016 Elsevier Inc.
539